Design has been a pursuit of humanity since day one. The practice of taking materials from the world around us and shaping them to create a useful function is one of the fundamental processes to have delivered us to the world we live in today.
The initial motivations for our ancestors were driven by the desire to facilitate meeting needs for provision of food, water and shelter – the fundamental requirements of survival. Shaping a hammer from a stone core, or using plant materials to build a shelter, were some of humanity’s earliest design enterprises.
With the perfection of concepts like the lever and pulley bolstering agricultural productivity, mechanisms such as the windmill soon emerged, enabling more complex functions to be considered and sparking an industrial revolution.
The evolutionary process of design flows such that innovations lead to innovations and, once basic needs are met, further experimentation is driven by some level of enjoyment gained from tapping into our innate desire and curiosity to keep exploring, optimising and doing better. This is what we know today as the pursuit of excellence.
For example, the imagination of the first wheel led to the innovation of the horse-drawn carriage, which, in relatively short time, led to the innovation of the motor car.
Sport from design
Following that glide path, it’s not difficult to see how humans, enjoying the comfort of plentiful food and warm houses, began to create sport out of design. This led to the development of hugely complex mechanisms like Formula 1 cars, made of thousands of components, each one of them a specialised evolution of a basic function, acutely focused on a specific objective.
The addition of sport to design activity is a significant point. With competition in the mix, the quality of a design is considered with a new scrutiny because an edge is gained by designing better than your opposition. This leads to some unique specialisations.
In any high-performance design, each innovation is undertaken with a focus on improving the previous function by a certain metric. When the designs are intended to bear loads, the metrics are strength, stiffness and weight.
With this, we enter the world of structurally efficient design.
What is structurally efficient design?
In motorsport, we need components to be strong enough to withstand their intended use without permanent, plastic deformation or damage. We need parts to be stiff and not flex excessively during operation.
The catch is we also need them to be light, because every excess gramme of weight carries a performance penalty, primarily in the form of a lap time increase.
Stiffness is a consideration that attracts focus in motorsport for very particular reasons. Testament to this is the suspension system, where excess deformation in the control arms or steering rack caused by high g lateral and longitudinal loading will dynamically alter the wheel’s camber and toe.
After spending many hours running countless simulations to dial in your kinematics, it would be tragic to have it ruined by an overly compliant suspension.
Stiffness quandary
If we design a part to be strong enough, it likely won’t be stiff enough. Conversely, make a part stiff enough without care to detail and it will be overly strong and too heavy.
To begin to untangle this problem, we need targets. Most structural parts will carry some compliance constraint, defined by their respective attribute group. This gives us a starting point to approach the design process.
Damian Harty, former CAE team leader at Prodrive and founder of Future Vehicle Systems, had the following thoughts to share on his approach: ‘In our suspension target definition, I used to ask what’s the smallest adjustment to the geometry we can make that the driver can measure? This was about one tenth of a degree for toe and a quarter, or half a degree for camber. So, that defined our compliance target under the maximal lateral loading we’d expect during a season.’
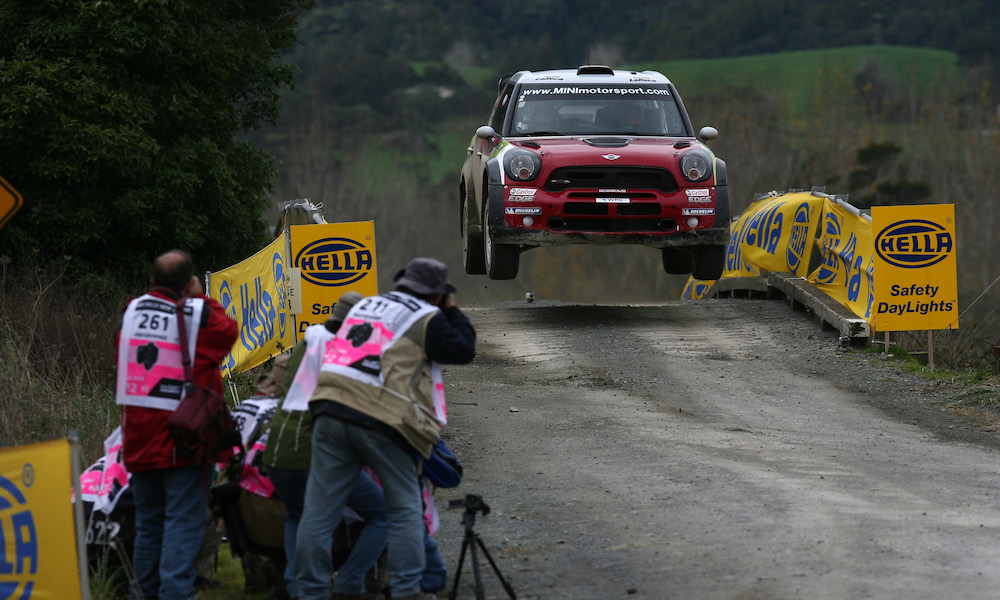
Compliance target
The first task to defining a compliance target into something useable is to have a sound understanding of the environment the part will be operating in, in terms of forces and moments in each degree of freedom.
In motorsport, unexpected loading events are almost a given, so must be accounted for. Defining nominal loading is straightforward enough, but in something like a suspension system or chassis structure we must also account for crashes, contact with another competitor, kerb strikes or other events that introduce abnormal loading into our components. The standard deviation of loading is therefore relatively high.
‘In our WRC project, we used to design the cars to withstand a vertical load of 11g, but we also wanted to be clear on what would break if we exceeded that, and what would happen as a result,’ recalls Harty. ‘By the time we were at those loads, the tyres were contacting the inner wheel well, and the armoured belly was in contact with the ground. The car could survive that, but seeing as much as 11g generally means the driver has done something quite wrong.’
Defining these upper limits is still very much a human process, where judgement, experience and data are part of the decision making. The idea is to design such that we have a reasonable confidence that we won’t see failure, even during abnormal events.
This is a sound philosophy, but can look quite different in its implementation across different component types.
Heavily loaded powertrain components, such as connecting rods, crankshafts and, to a lesser extent, gearbox and driveshaft components, all must withstand very high peak loads. However, as the combustion process is reasonably repeatable, the standard deviation of these loads is way less than that of wheel loads.
Chasing efficiency
The objective is to achieve high stiffness while using the minimum amount of material possible. This is where the ‘efficient’ element of structural design comes into focus.
Structurally efficient design is an extremely interesting domain. It can be distilled into the following considerations: 1) robust material selection; 2) design that mitigates localised stress concentrations in the part with filleted edges and no abrupt section changes; 3) optimisation of the stress distribution through the part; 4) consideration of the section modulus to maximise bending stiffness relative to the volume of material used.
Clearly, then, the choice of material for a component is a meticulous process.
Stiffness at the material level is often evaluated through what is called the
specific modulus. This relates the part’s stiffness (Young’s modulus) to its density. Interestingly, the most commonly used high-performance engineering materials – aluminium, steel and titanium alloys – all have a similar stiffness modulus.
This means for a given weight, they are all just about as stiff as each other. There are no advantages to be gained there, apparently. So, the appropriate material choice isn’t immediately obvious without further consideration.
Evaluating strength with respect to density is another way to filter the good from the bad. Here, specific strength is our metric. A higher specific strength means less material is needed for a given part strength, so initially we want materials to have both high specific strength and specific modulus.
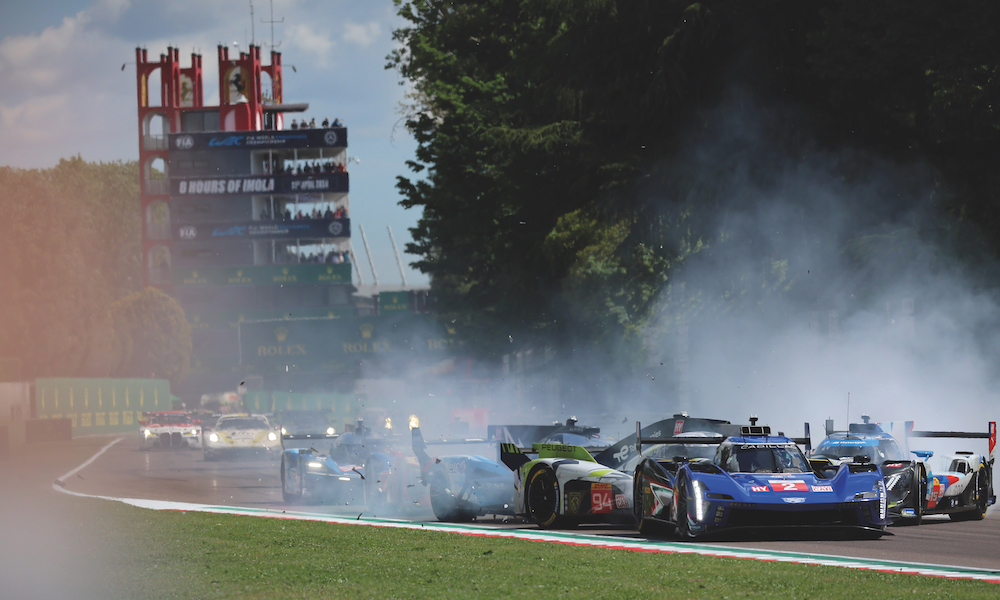
Material choice
The high strength of titanium alloys like Ti-6AL-4V is attractive, but it loses out to steel grades such as AISI 4340 on specific modulus. An aluminium alloy such as 7075-T6, on the other hand, performs well in stiffness and strength, comparable to both steel and titanium, but falls short in fatigue resistance, elongation and toughness. This means it bends less before failing and can withstand fewer loading cycles.
Carbon fibre stands out above metal alloys for some of these metrics, so can be a strong choice for applications where loading modes are well understood and relatively simple. However, unlike metal alloys, which are isotropic and exhibit the same strength in all directions, anisotropic composites like carbon fibre have mechanical properties that vary with loading direction.
This makes a material challenging to apply in complex loading scenarios, and its low elongation and toughness means failure is often catastrophic when yield is exceeded.
Special mention here should be given to some of the more exotic alloys, such as Al-Li (aluminium-lithium), Al-Be (aluminium-beryllium) and MMC (metal matrix composites), all of which offer some very attractive properties, but are generally tightly controlled by regulations due to their huge expense (or, in Al-Be’s case, outright banned because of its toxicity).
It’s not hard to see how complex the matrix of considerations is to pick the right material for a job.
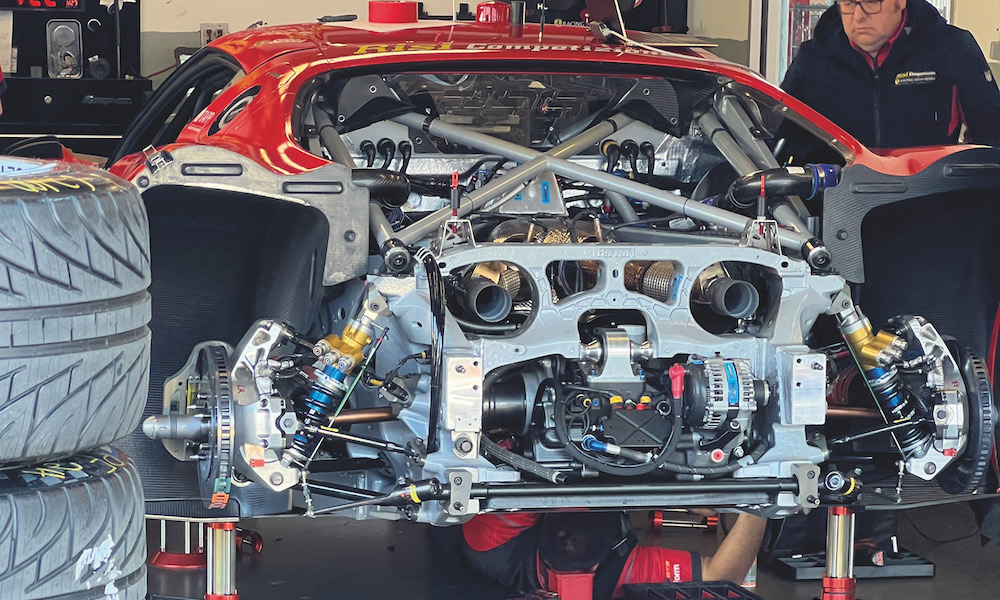
Stress and strain
The loading experienced up to yield stress can be simplified as the linear strain region, where the relationship between stress and strain is approximately linear. With continued strain, it enters the realm of plastic deformation, where the relationship between stress and strain becomes highly non-linear.
These distinct properties form a lineation in material behaviour, and we ideally want our upper design load to sit right at that transition of linear to nonlinear response.
Materials and their stress / strain responses are fascinating, but component design is the realm where it all starts to become a little more tangible.
Joining our components together to form the structure is clearly the most pressing concern and, while packaging and kinematic constraints will certainly dictate some of the final form, there is a huge amount to be said for craftsmanship.
One wonders if the fact that pretty, aesthetically pleasing structural designs are often the most efficient load bearing shapes is purely a coincidence, or an innate feeling we all have for good and sound design.
Sharp edges give rise to sharp stress gradients, so fillets and smooth edges and transitions are a designer’s best friend. That’s elementary, but further refinement requires a trained eye, and a particular inspiration.
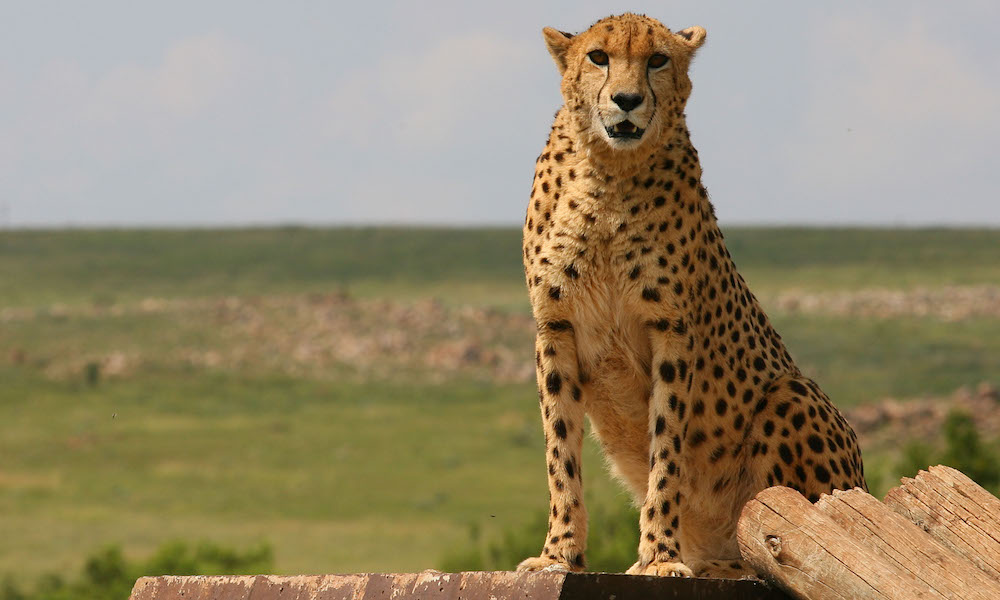
Nature’s gift
The field of biomimetics recognises that nature has some truly spectacular engineering solutions. Bones of animals feature trabecular tissue, which is specifically present to increase the stiffness and strength of bones without largely impacting the mass.
Bones also provide a brilliant observation of maximising a geometric property called the section modulus, which provides a metric of a form’s ability to resist bending stress.
A high section modulus is achieved by placing material away from the neutral axis, where the bending stress is zero, raising the moment of inertia and, in turn, the stiffness for a given quantity of material.
Applying this to motorsport engineering is the reason we have larger diameter tubes in roll cages, and why aluminium parts are generally larger section than an equivalent steel part. A great practical demonstration of the effect of an increased section modulus can be found in the steering rack.
A steering rack can be simplified as a bar inside a tube, supported in two places. The bar (rack) has teeth cut into it to allow the pinion gear to move it back and forth as the steering column rotates.
‘As the suspension articulates, there is an appreciable bending moment on it that makes the rack flex vertically, in a meaningful way,’ explains Harty. ‘When we were looking at compliance on the BMW Mini Countryman project [at Prodrive], we rotated the rack to give us the stiffer side of the bar working against the bending moment. It worked really well, and just seemed so obvious when we looked at the model.’
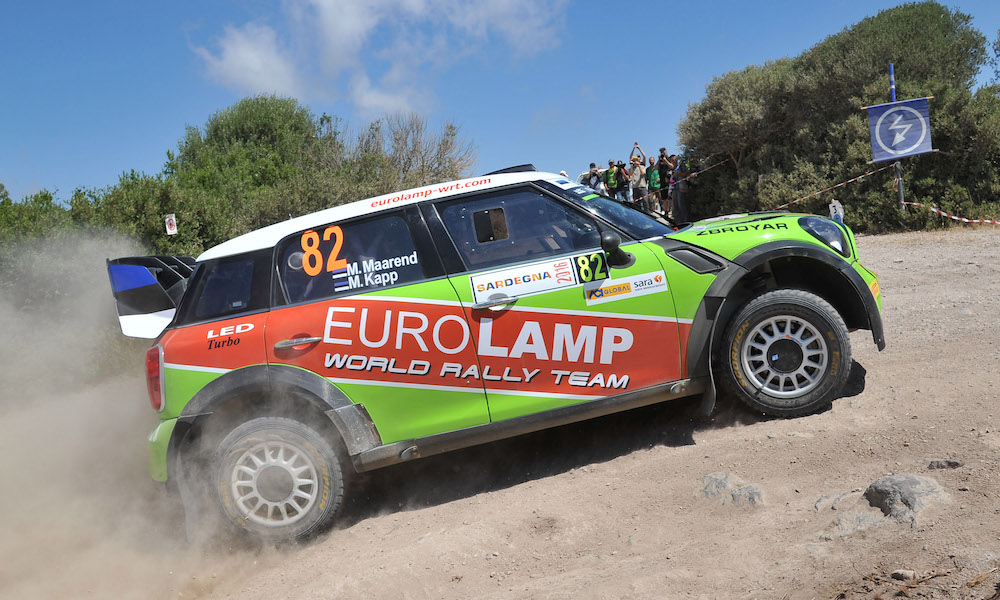
Validation time
With such time and focus on achieving structurally efficient design, painstakingly selecting the correct alloys and designing elegant part geometries, we of course need methods of validating the resulting component.
In earlier times, performing structural analysis was a slow process, but it has now been revolutionised by simulation and computing power.
Finite element analysis (FEA) tools, for example, have advanced leaps and bounds in both ease of use and integration into the design process. They use mathematical models of material behaviour and, in the linear strain range at least, provide quick, relatively simple and accurate predictions of how a material will behave.
Results from the FEA are fed back to the designer in very short time to allow modification of the design based on stress concentrations and overloaded areas. This iterative approach to design has been in practice for decades and, while there have been efficiency improvements to workflows and methodologies, the basic principles have remained static.
Additionally, 3D printing and metal sintering techniques have allowed some very interesting and previously unachievable geometries to be developed.
Validation revolves around gathering physical data from real-world testing to correlate the FEA to observations on prototype parts from tests in a lab setting on test rigs or running the part on a real vehicle on an accelerated durability test. By validating FEA predictions with empirical data, engineers can identify discrepancies and refine their models to improve accuracy. This iterative process ensures the final design meets performance targets, ultimately leading to more reliable and robust components.
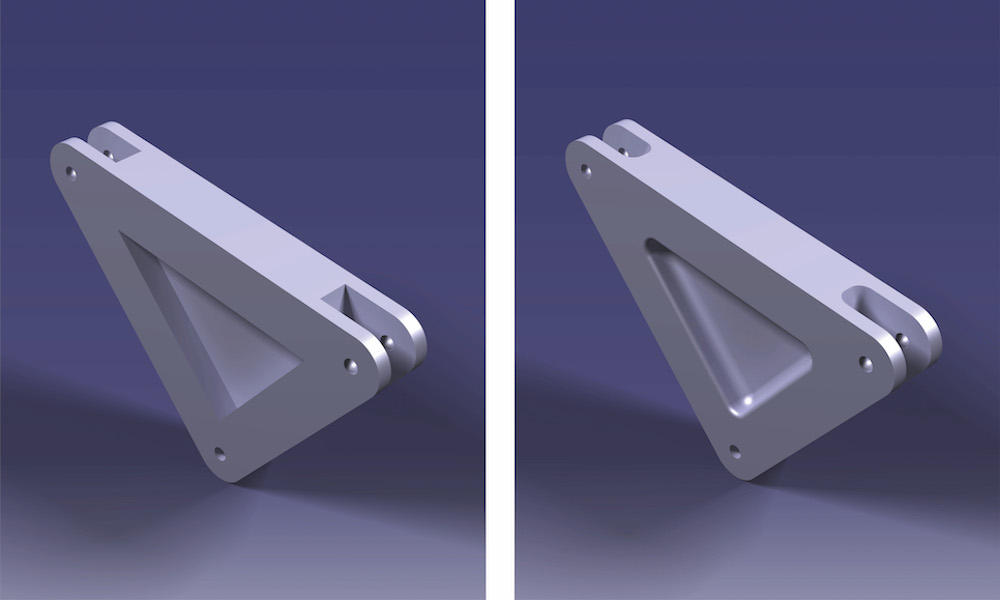
What the future holds
The future of structurally efficient design in the motorsport environment will be significantly influenced by advancements in materials science and manufacturing techniques. Part of this revolution will be through emerging technologies such as metamaterials and nanomaterials.
Metamaterials are engineered materials, which exhibit properties not found in naturally occurring substances. They have been an area of intense research, partially unlocked through improvements in additive manufacturing technology such as selective laser melting (SLM), which allows for the creation of complex, periodic structures with extremely high precision.
Similarly, nanomaterials are making waves. By reducing the grain size of materials like titanium and aluminium, researchers have significantly increased their yield strengths. Carbon nanotubes (CNTs), when integrated into composites like carbon fibre (CFRP), improve stress distribution and provide substantial benefits in terms of fatigue resistance and crack mitigation.
These cutting-edge materials share the common goal of enhancing the strength and stiffness of components while, at the same time, minimising weight. Although there are still challenges to overcome, the future looks promising.
The pursuit of structurally efficient design is a dynamic and evolving field. From the historical advancements in basic mechanical principles to the sophisticated integration of modern materials and computational techniques, the journey is a remarkable one.
Continuous improvements in material science, coupled with advancements in simulation and optimisation algorithms, promises a future where designs are not only lighter and stronger but also more adaptable and resilient. If there are benefits to be found, we can be sure motorsport will find them.